Light & Coherence part 2: Spatial Coherence (and the Double Slit Experiment)
Summary
TLDR本视频深入探讨了空间相干性及其对光性质的影响。通过荷兰希尔弗瑟姆的洛伦兹池塘实例,阐释了波的空间相干性如何随距离源点增加而增强。视频还通过数值模拟展示了波的空间相干性发展过程,并定量分析了相干区域与波源几何尺寸的关系。此外,视频对托马斯·杨的双缝实验进行了讨论,指出了科学传播中对实验配置的简化和误解。最后,通过双缝实验和光栅实验,展示了空间相干性在光的干涉和频谱分析中的作用,引发了对光的波动性和量子性质的深入思考。
Please replace the link and try again.
Q & A
什么是空间相干性?
-空间相干性是指光波在空间分布上的有序性和一致性。当光波的相位在一定区域内保持一致时,我们可以说这些光波在该区域内具有空间相干性。
视频中提到的Hilversum的池塘是如何展示空间相干性的?
-视频中的Hilversum池塘通过喷泉产生的水波来形象地展示空间相干性。喷泉附近的水面波动较为混乱,但随着距离的增加,水波逐渐变得有序并朝同一方向移动,展示了空间相干性随距离增加而增强的现象。
视频中提到的Nils Berglund的模拟实验是如何帮助理解波的空间相干性的?
-Nils Berglund的模拟实验通过展示15个随机位置的波源发出的波如何在时间和空间上发展,帮助我们更详细地理解波是如何逐渐获得空间相干性的。实验中波源发出的波最初是混乱的,但随着距离的增加,波的模式变得更加有序,这与实际池塘中观察到的现象相似。
波的空间相干性与波的几何特性是如何关联的?
-波的空间相干性可以通过相干面积来表征,该面积与波源距离的平方、波长的平方成正比,与波源直径的平方成反比。这个关系简化了实际情况,但提供了一个有用的公式来估算相干区域的大小。
Thomas Young的双缝实验是如何利用空间相干性的?
-Thomas Young的双缝实验通过使用单色光和两个非常接近的缝隙来展示空间相干性的效果。只有当光源产生的相干面积足够大,以至于覆盖了两个缝隙的整体区域时,才能在屏幕上观察到明显的干涉条纹模式。
视频中提到的关于Antares星的空间相干性计算结果是什么?
-根据视频中的计算,Antares星的光到达地球后,其空间相干区域大约是2.3平方米。这意味着尽管Antares是一个巨大的恒星,其发出的光在地球上表现出几乎均匀的相干性。
为什么我们需要空间相干性来进行双缝实验?
-进行双缝实验需要空间相干性,因为只有当来自两个缝隙的光波在空间上具有固定的相位关系时,它们才能在重叠区域形成干涉条纹。如果光波在缝隙区域内没有相位关系,就无法形成明显的干涉模式。
视频中提到的关于光的经典视角和量子力学视角有何不同?
-经典视角主要关注光作为电磁波的传播、散射、衍射等现象,而量子力学视角更侧重于光的发射和吸收过程中的能量包转移,以及这些过程中的量子化特性。
视频中提到的关于光的波粒二象性是如何理解的?
-光的波粒二象性是指光既表现出波动特性,如干涉和衍射,也表现出粒子特性,如光电效应和康普顿散射。视频中提到,尽管光的相互作用过程是量子化的,但这并不一定意味着光本身就必须被看作是能量的离散包。
视频中提到的关于光的相干面积公式是如何推导的?
-相干面积公式是基于波源几何形状和波的干涉条件推导出来的。考虑一个扁平的圆形波源,只有来自波源最外侧的波在远离中心轴的某一点上保持同相。随着距离中心轴的增加,这些波逐渐失去同相性。定义一个距离Y,使得两点间的相位差小于一个可接受的值,这个距离Y与波长成正比,与波源距离和波源大小成反比。
视频中提到的关于光的传播方向对空间相干性的影响是什么?
-光的传播方向对空间相干性有重要影响。当光波从不同的波源发射出来时,它们的传播方向会随着距离的增加而逐渐对齐。这种对齐导致了波的相位关系变得一致,从而在一定距离外形成了空间相干性。
视频中提到的关于光的干涉和衍射现象有什么区别?
-干涉现象是指两个或多个相干光波在空间某点相遇时,由于相位差引起的光强增强或减弱的现象。而衍射是指光波遇到障碍物或通过狭缝时,波前发生弯曲和扩散的现象。在双缝实验中,衍射和干涉同时发生,共同作用形成了复杂的干涉条纹模式。
Outlines
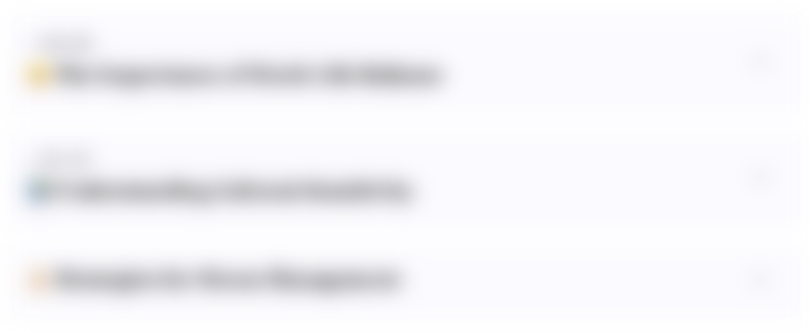
此内容仅限付费用户访问。 请升级后访问。
立即升级Mindmap
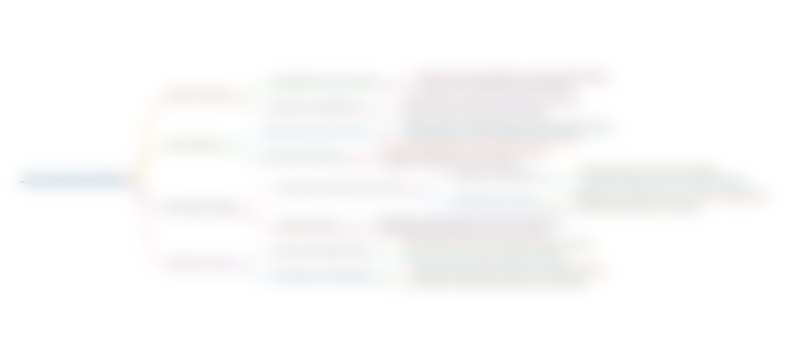
此内容仅限付费用户访问。 请升级后访问。
立即升级Keywords
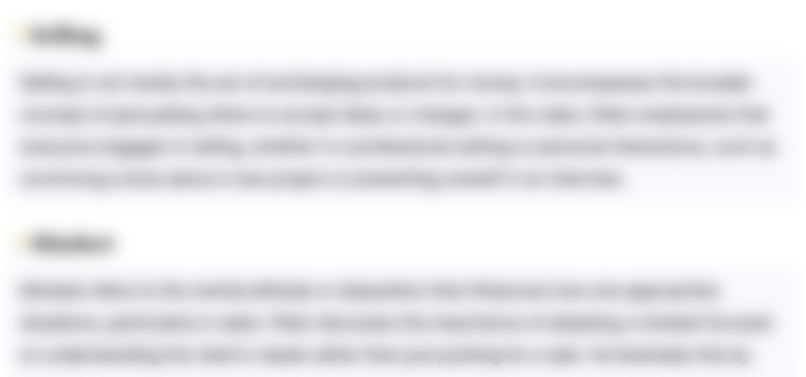
此内容仅限付费用户访问。 请升级后访问。
立即升级Highlights
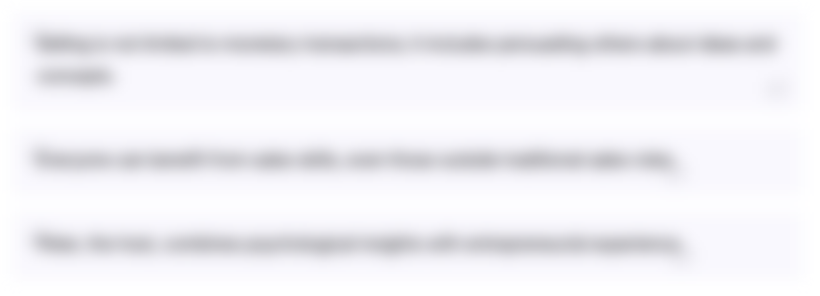
此内容仅限付费用户访问。 请升级后访问。
立即升级Transcripts
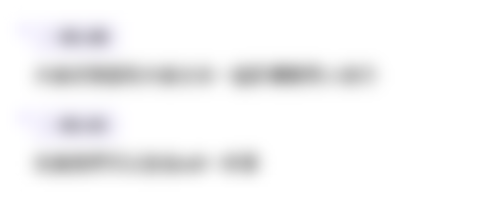
此内容仅限付费用户访问。 请升级后访问。
立即升级浏览更多相关视频
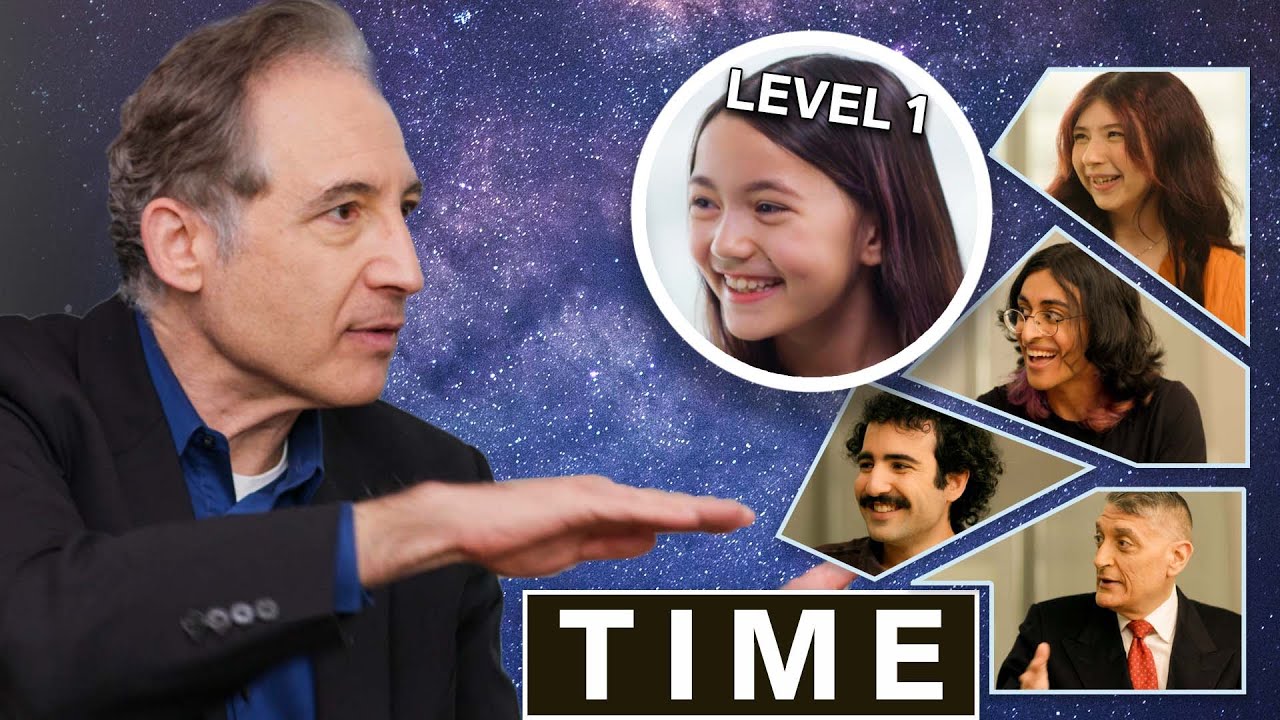
Theoretical Physicist Brian Greene Explains Time in 5 Levels of Difficulty | WIRED

OpenAI shocks the world yet again… Sora first look

CCIR - Quantum Physics: Information, Foundations And Gravity - Lecture Session #2

CCIR - Quantum Physics: Information, Foundations And Gravity - TA Session #1
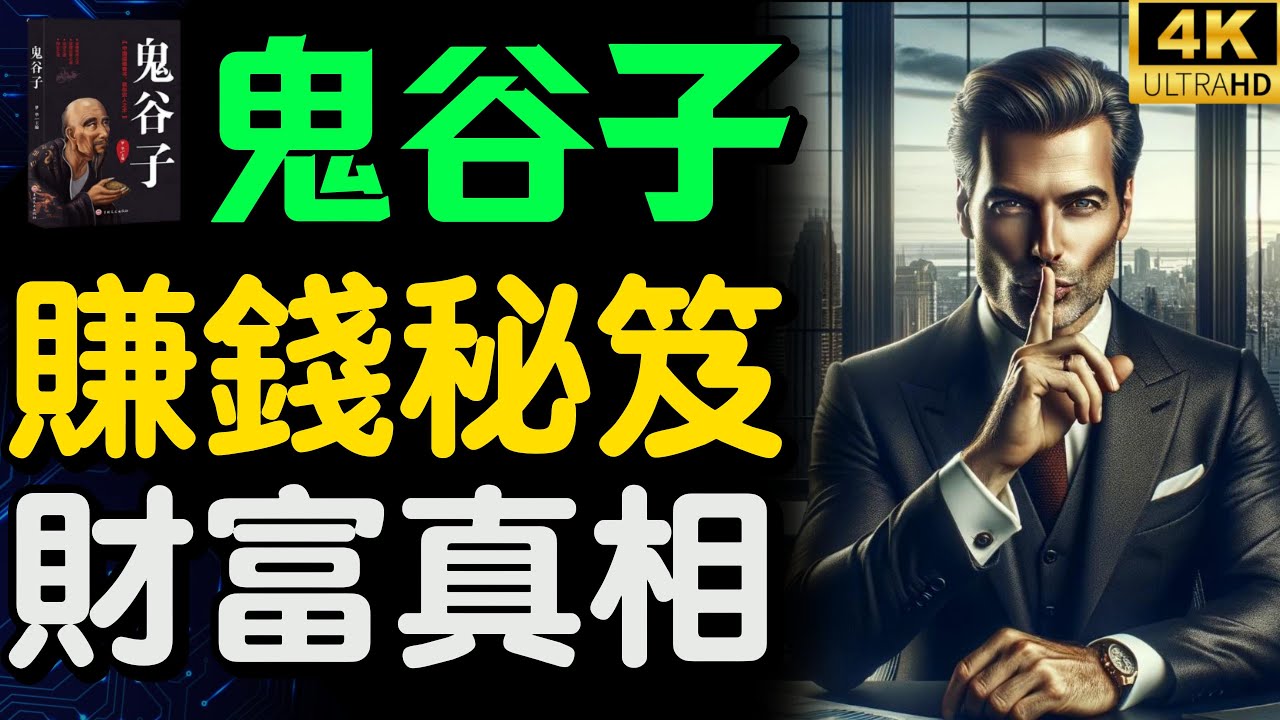
鬼谷子賺錢秘籍 | 揭秘財富真相 | 沒有誰的錢是賺不到的?【財之道】富人思維 財之道 目標設定 財商 賺錢 破局思維 財富自由 認知 財商知識 個人成長 開悟覺醒 反內耗 @moneyrules8
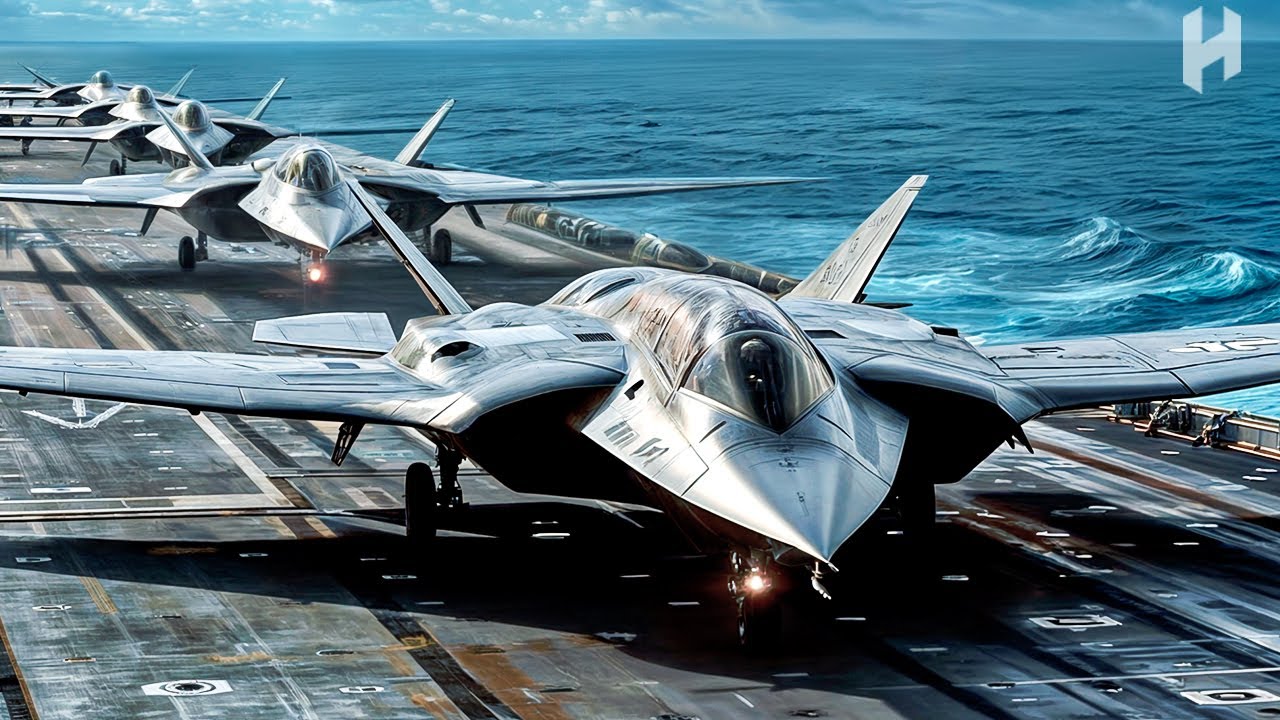
Russia’s NEW $600 Million Attack Fighter Can ANIHILATE Every US Air Force Jet

Why C.S. Lewis Is as Influential as Ever
5.0 / 5 (0 votes)