Light & Coherence part 1: Temporal Coherence
Summary
TLDRThis video demonstrates an experiment using a Michelson interferometer to explore light coherence properties. The speaker compares spatially coherent white light and laser diode light in both spontaneous and stimulated emission modes. Through visualizing interference patterns, the experiment highlights how coherence lengths vary, from the short coherence of white light (~1 micron) to the long coherence of lasing light (several meters). The video introduces key concepts of temporal and spatial coherence and sets the stage for deeper exploration into how interference and coherence affect energy distribution in space.
Takeaways
- 😀 The Michelson interferometer setup consists of two mirrors, with one placed on a translation stage to vary the path length.
- 😀 A spatially coherent white LED source was used in the first experiment, comprising a white LED, pinhole, collimation lens, and aperture.
- 😀 The white light source produces a uniform beam that appears parallel over long distances due to spatial coherence.
- 😀 Interference patterns are observed when the arms of the interferometer are of equal length, with the accuracy required being just a few wavelengths.
- 😀 Temporal coherence is a key factor, and interference patterns decay quickly as the path length difference increases, especially with broad-spectrum light like white light.
- 😀 Different wavelengths of light (e.g., blue and red) produce interference patterns with varying spacings, with blue having the smallest and red the largest.
- 😀 The coherence length of white light is approximately 1 micrometer (2 wavelengths), which is very short.
- 😀 When using a laser diode at low current, the diode operates like an LED, showing spontaneous emission and a coherence length of about 4.5 microns.
- 😀 Increasing the current of the laser diode causes stimulated emission, resulting in a significant increase in coherence length (up to several meters).
- 😀 The coherence length of light from stimulated emission is much longer than spontaneous emission, showing a difference of up to a million times.
- 😀 The experiment demonstrates how coherence length can vary greatly depending on whether the light source is operating in spontaneous or stimulated emission mode.
- 😀 The presenter promises to continue the discussion in a second video, focusing on spatial coherence and its impact on energy distribution in space.
Q & A
What is the main purpose of using the Michelson interferometer in the experiments described?
-The Michelson interferometer is used to study the coherence properties of light sources, specifically focusing on temporal coherence. The setup allows the observation of interference patterns to explore how light behaves when path lengths are varied.
What is meant by spatially coherent light, and how is it created in the experiment?
-Spatially coherent light refers to light where the phase and intensity are uniform across the beam's cross-section. In the experiment, this is achieved by using a white LED, a pinhole, a collimation lens, and an aperture, which ensures the beam is parallel over long distances.
Why is it difficult to observe an interference pattern with white light?
-It is difficult because white light is spectrally broad, meaning it contains many different frequencies (colors). As the path length difference increases, the various frequencies quickly lose coherence with each other, making the interference pattern fade unless precise matching of the path lengths is achieved.
What is the significance of the interference pattern spreading across the CCD sensor?
-The spreading of the interference pattern across the CCD sensor occurs because the mirrors in the interferometer are slightly tilted. This tilting allows the temporal coherence of the light to be observed as a spread-out pattern, enabling the visualization of how different wavelengths of light lose coherence at different distances.
How is the coherence length of the white light determined from the interference pattern?
-The coherence length of the white light is estimated by analyzing the interference pattern. By converting the pattern into an intensity plot and examining the spacing between the peaks of the interference, the coherence length can be approximated. In this case, it was found to be about 1 micrometer.
How does the behavior of the laser diode change when operated in spontaneous emission mode versus stimulated emission mode?
-In spontaneous emission mode (low current), the laser diode behaves like a normal LED with a relatively short coherence length. In stimulated emission mode (higher current), the diode produces a narrow spectral distribution, resulting in a much longer coherence length and more stable interference patterns.
What was the observed effect on the interference pattern when the current of the laser diode was increased?
-When the current was increased to the point where the laser diode began to lase, the interference pattern became much clearer and more stable. The coherence length increased dramatically, and the interference was observable even when the path length difference was several meters.
How does the coherence length of white light compare to that of the laser diode in stimulated emission mode?
-The coherence length of the white light (about 1 micrometer) is much shorter compared to the laser diode in stimulated emission mode, which can maintain coherence over distances of several meters—approximately a million times longer.
Why is it important to carefully control the current when using the laser diode in stimulated emission mode?
-Careful control of the current is important to ensure stable operation of the laser diode, which is necessary for achieving a long coherence length and a clear, stable interference pattern. Too much variation in current could cause instability in the laser's emission.
What will be discussed in the next video, according to the script?
-The next video will delve into spatial coherence in more detail and continue the discussion on how phenomena related to coherence and interference can create a non-uniform distribution of energy in space.
Outlines
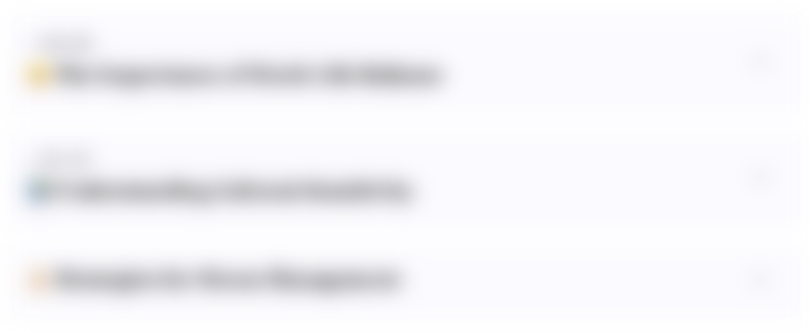
このセクションは有料ユーザー限定です。 アクセスするには、アップグレードをお願いします。
今すぐアップグレードMindmap
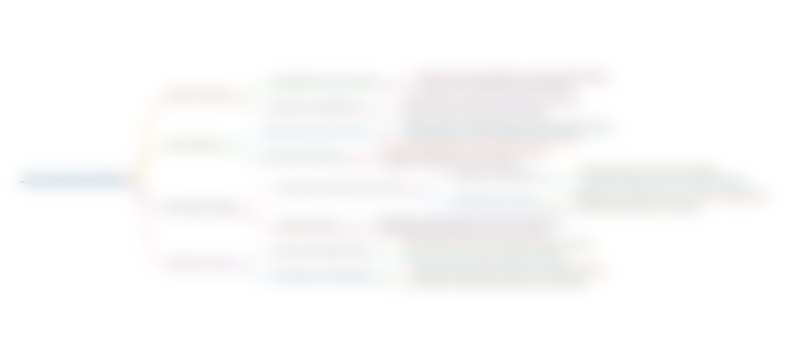
このセクションは有料ユーザー限定です。 アクセスするには、アップグレードをお願いします。
今すぐアップグレードKeywords
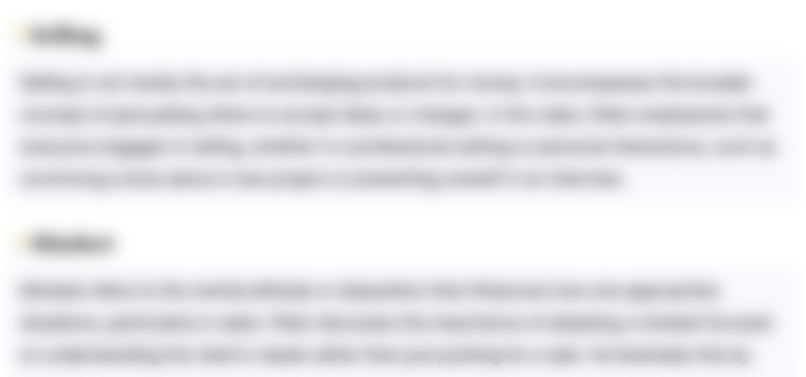
このセクションは有料ユーザー限定です。 アクセスするには、アップグレードをお願いします。
今すぐアップグレードHighlights
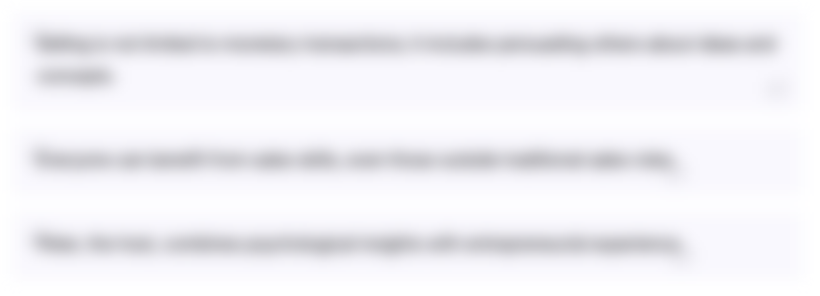
このセクションは有料ユーザー限定です。 アクセスするには、アップグレードをお願いします。
今すぐアップグレードTranscripts
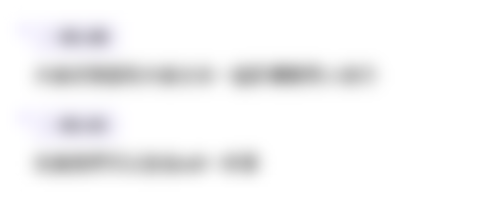
このセクションは有料ユーザー限定です。 アクセスするには、アップグレードをお願いします。
今すぐアップグレード5.0 / 5 (0 votes)