The Map of Quantum Computing - Quantum Computing Explained
Summary
TLDRThis video script offers an insightful exploration into the world of quantum computing, tracing its evolution since the 1980s and highlighting the recent surge in industry growth. It simplifies complex concepts such as superposition, entanglement, and interference, which are fundamental to understanding how quantum computers operate differently from classical ones. The script delves into various quantum computing models, including the gate model, adiabatic quantum computing, and topological quantum computing, each with unique approaches to harnessing qubits. It also discusses the practical challenges of building quantum computers, such as decoherence and scalability, and touches on potential applications in optimization, machine learning, and quantum simulation. The video is sponsored by Qiskit, an educational resource for those eager to learn more about quantum computing.
Takeaways
- 🌟 The quantum computing industry has seen significant growth since the 1980s, with many companies investing heavily in the development of advanced quantum computers.
- 🚀 Quantum computers operate differently from classical computers, leveraging principles like superposition, entanglement, and interference to solve problems that are difficult or impossible for classical computers.
- 📊 Qubits, the fundamental units of quantum information, can exist in multiple states simultaneously, unlike classical bits which are either 0 or 1.
- 🔗 Entanglement is a key quantum phenomenon where qubits become interconnected, affecting the state of the entire system when any single qubit is altered.
- 🌐 Superposition allows qubits to exist in a state that is a combination of 0 and 1, influencing the probability of the output when measured.
- 🔍 Quantum algorithms, such as Shor's algorithm, can solve complex problems like integer factorization more efficiently than classical algorithms, potentially impacting security protocols.
- 🛠 Quantum computers have a wide range of potential applications, including optimization, machine learning, financial modeling, and quantum simulation for material science and drug development.
- 💡 Quantum simulation is highlighted as a particularly promising application, offering exponential speedup over classical computers for simulating quantum systems.
- 🌐 Quantum computing models include the gate model, adiabatic quantum computing, quantum annealing, and topological quantum computing, each with unique approaches to manipulating qubits.
- 🔬 Physical implementations of qubits vary widely, with superconducting qubits, quantum dots, linear optical quantum computing, trapped ion, color centers, and neutral atoms in optical lattices among the leading methods.
- 🔄 The challenges of building quantum computers include decoherence, noise, and scalability, which are being addressed through techniques like quantum error correction and advanced engineering.
Q & A
What is the main focus of the video 'Map of Quantum Computing'?
-The video aims to provide a comprehensive overview of different types of quantum computing, how they work, and why there is significant investment in the quantum computing industry.
What are the three fundamental concepts needed to understand how quantum computers work?
-The three fundamental concepts are superposition, entanglement, and interference, which are the building blocks of quantum computing.
How do quantum bits, or qubits, differ from classical bits in terms of their states?
-While classical bits can only be in one state at a time (0 or 1), qubits can be in a superposition state, allowing them to be in a combination of 0 and 1 states simultaneously.
What is the significance of entanglement in quantum computing?
-Entanglement allows qubits to become part of one large quantum state, making them interdependent. Changes to one qubit can affect the probability distribution of the entire system, which is crucial for quantum computing's power.
How does the concept of interference play a role in quantum computing?
-Interference, through the constructive and destructive addition of wavefunctions, influences the probabilities of different states in a quantum computer. It is used in quantum algorithms to increase the likelihood of the correct answer and decrease the likelihood of incorrect ones.
What is Shor's algorithm and why is it significant in the field of quantum computing?
-Shor's algorithm is a quantum algorithm that can efficiently find the factors of large integers. It is significant because it demonstrated the potential of quantum computing to solve problems considered intractable on classical computers, such as integer factorization, which has implications for cryptography.
What is quantum complexity theory and how does it relate to the efficiency of quantum algorithms?
-Quantum complexity theory is a subfield that categorizes algorithms based on their efficiency on quantum computers. It helps in understanding how much harder it is to solve a problem as the problem size increases and classifies problems like factorization into complexity classes, showing which are more efficiently solvable by quantum computers.
What are some potential applications of quantum computers beyond cryptography?
-Beyond cryptography, potential applications include quantum simulation for studying chemical reactions or material properties, optimization problems, machine learning, financial modeling, weather forecasting, and climate change research.
What are some of the challenges faced in building a practical quantum computer?
-Challenges include decoherence, where information leaks due to interaction with the outside world, noise from various sources that can cause errors, and scalability issues as the number of qubits increases, requiring more complex control and measurement systems.
What is the difference between the gate model and adiabatic quantum computing?
-The gate model involves a sequence of quantum gates applied to entangled qubits to perform computations, while adiabatic quantum computing leverages the natural tendency of physical systems to move towards the minimum energy state to solve problems, with the solution being the lowest energy state of the system.
Can you explain the concept of quantum error correction and its importance?
-Quantum error correction is a scheme that uses multiple entangled qubits to represent a single noise-free qubit. It is important because it helps create fault-tolerant quantum computers by protecting against decoherence and noise, which are major obstacles in building practical quantum computers.
What are some of the physical implementations of qubits that are being explored in quantum computing?
-Some of the physical implementations being explored include superconducting qubits, quantum dot or silicon spin qubits, linear optical quantum computing with photons, trapped ion quantum computers, color center or nitrogen vacancy quantum computers, and neutral atoms in optical lattices.
What is the current state of quantum computers in terms of their ability to solve real-world problems?
-As of the video's information, quantum computers have not yet reached the stage where they can consistently solve real-world problems better than classical computers. Current quantum computers are still in the development phase, and much research is focused on overcoming technical challenges and scaling up the technology.
Outlines
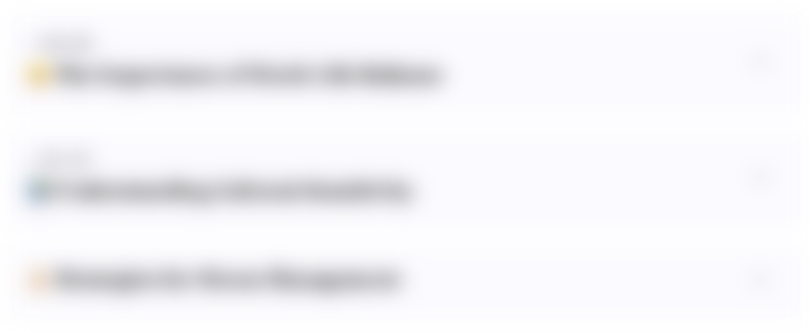
This section is available to paid users only. Please upgrade to access this part.
Upgrade NowMindmap
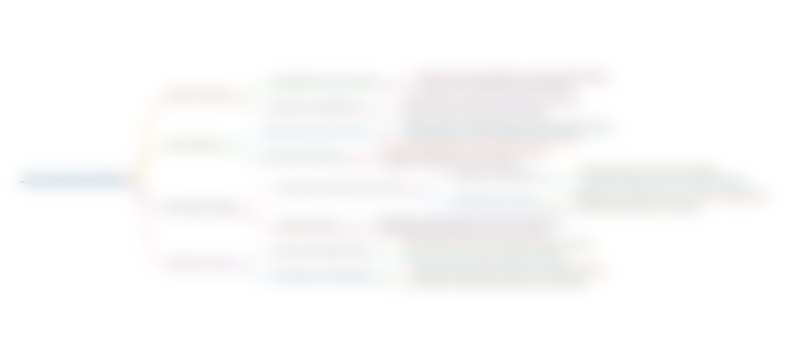
This section is available to paid users only. Please upgrade to access this part.
Upgrade NowKeywords
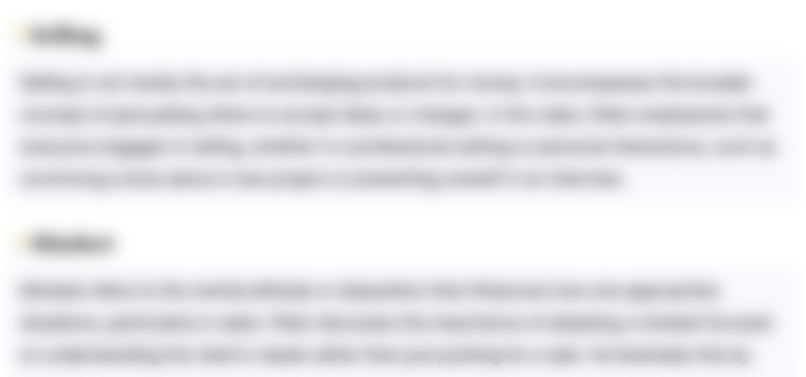
This section is available to paid users only. Please upgrade to access this part.
Upgrade NowHighlights
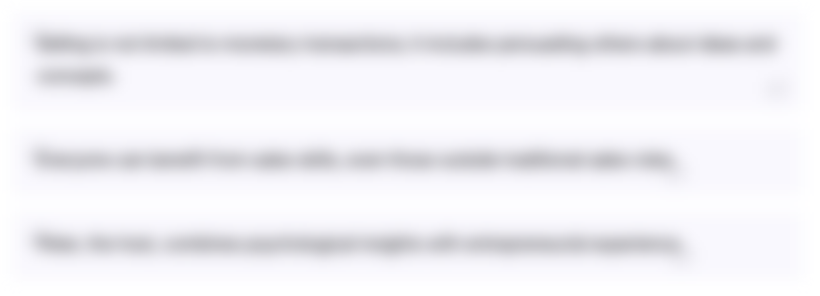
This section is available to paid users only. Please upgrade to access this part.
Upgrade NowTranscripts
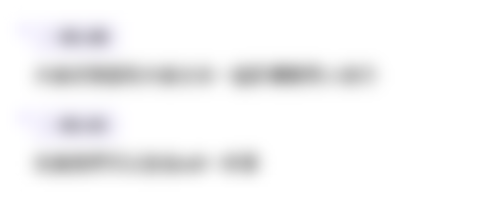
This section is available to paid users only. Please upgrade to access this part.
Upgrade NowBrowse More Related Video
5.0 / 5 (0 votes)